Impact
of Earthquakes
|
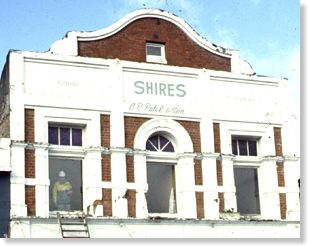
Damage from the 19 February 1990 Weber earthquake. This building in the main street of Dannevirke was condemned, and new retail premises were built. V.E. Neall
|
|
The impact of a large earthquake on land and buildings is usually
very obvious. There are consistent relationships between the amount
of damage and the properties of both buildings and the ground on
which they are sited.
During an earthquake the ground surface moves in all directions.
The most damaging effects on buildings are usually the horizontal
movements because most structures are designed to withstand vertical
gravity loads.
Ambrose and Vergun (1990) identify the general
effects of earthquakes as:
1. Direct movement of structures.
The structure is attached to the ground; when the ground moves,
so does the structure. This destabilises the structure by shaking.
2. Ground surface faults.
When the fault movement propagates to the surface during an earthquake,
cracking and slumping occurs, which can damage roads and railways,
fence lines and structures. If the ground surface settles during
an earthquake, it may become flooded. The earthquake may trigger
landslides. In sandy soils the ground may flow like a liquid (liquefaction)
and buildings may sink below ground level.
3. Tsunami. Ground
movements caused by earthquakes can set up large waves on overlying
bodies of water. These waves may travel hundreds of kilometres,
at hundreds of kilometres an hour and may reach heights up to
40 m.
4. Earthquakes can damage dams, trigger small scale tsunamis
in reservoirs and may sever pipelines, all of which will cause
flooding, fire, gas explosions etc.
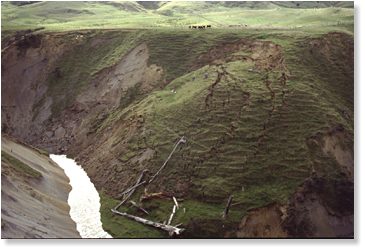
Landsliding created by 19 February 1990 Weber earthquake, damming a stream and breaking a hillside with future failure planes. Photo from Dannevirke-Weber Road. V.E. Neall |
|
|
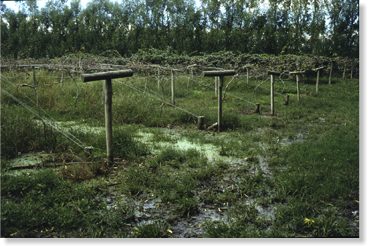
Kiwifruit orchard that subsided 1.5 m, near Thornton, due to faulting during the 1987 Edgecumbe earthquake. This lowered part of the orchard below the water table and drowned the kiwifruit. V.E. Neall |
|
Direct movement of structures Structures will be shaken
in all directions during an earthquake. The force effect caused
by this motion is generally directly proportional to the dead weight
of the structure, which also bears on the dynamic response of the
structure. Two other factors that will influence the structure's
response are:
1. The structure's resonance (determined by
the mass, stiffness and size of the structure; Fig. 14).
2. The structures efficiency in energy absorption (determined by
the elasticity of the structure, stiffness of supports, number of
separate moving parts etc.).
The relationship between the resonance of the earthquake and the
structure is a major concern. This relationship is shown as spectrum
curves (Fig. 14). Earthquakes have their major direct
force effect on structures with short resonance (e.g. small or squat
buildings, structures with stiff lateral resistive systems). Resonance
of tall towers and multi-storey buildings may be so long that different
parts of the building move in opposite directions simultaneously
(Fig. 15)
All structures have a natural resonance and if a building has a
frequency similar to that of the seismic waves then the movement
of the building is amplified by resonance and severe damage may
result. Different parts of a single building may have different
frequency responses, leading to differential damage, e.g. collapse
of a tower while a low storey part of the same building is undamaged.
Earthquakes and bedrock Figure
16.
Earthquakes
behave differently depending on the type of bedrock or sediments
they pass through. Figure 16 shows recording of horizontal
ground shaking for a variety of ground conditions. There is a general
relationship between earthquake intensity and acceleration for different
bedrock conditions. Significantly, these bedrock conditions represent
the foundation conditions for structures. Foundation material comprising
soil or landfill is likely to produce 3 times the ground acceleration
than firm bedrock (Fig. 17; Rahn 1986). The 1775 Lisbon,
Portugal earthquake destroyed the half of the city which was underlain
by soft Tertiary strata.
Figure
17.
Weak
earthquake tremors can be amplified as they pass through water-logged
muds, or clays which contain large amounts of water in their mineral
structure. An earthquake in Mexico on 19-20 September 1985 (epicentre
offshore, 400 km southwest of Mexico City), killed nearly 20,000
people in Mexico City. This city is built on montmorillonitic clays,
which can increase their water content by 350 - 400 %. These clays
can behave as if they are fluids. Near to the epicentre, which was
sparsely populated, damage was minimal. Major populations centres
close to the epicentre were badly affected, but damage decreased
sharply away from the epicentre.
At Mexico City the peak acceleration of the earthquake corresponded
to an estimated return period of 50 years. However, as the earthquake
shock wave passed thorough the clays beneath the city they were
amplified 6 times. Surface waves, just like ocean waves, crossed
back and forth across the basin for nearly three minutes. The wavelengths
of these waves (50 m) closely matched the dimensions of the bases
of many of the city's buildings. Peak accelerations for the surface
seismic waves matched those for the buildings which resonated, amplifying
the shaking of the buildings sufficiently to cause them to collapse.
Liquefaction
Liquefaction occurs when shear waves distort the structure of soils,
causing a soil to suddenly collapse. Soil comprises grains which
are in contact with one another. Below the water table the spaces
(voids) between these grains are filled with water. As long as the
grains stay in contact the soil will retain its strength. However,
if pressure exceeding the weight of the soil is put upon the water
in the voids the soil dilates, and the soil turns into a slurry
which behaves like a fluid and has virtually no bearing strength.
This process can happen very quickly and structures built on top
of soil which behaves in this way will sink into the slurry.
Liquefaction can be triggered by earthquakes. Shear waves disrupt
and reorganise the soil particles which decreases void space and
thus increases pore pressure with pressure increasing with each
passing shock. The size of the grains in the soil is an important
factor. Fine-grained soils, with a large proportion of silt and
clay, are less likely to liquefy because the capillary water tension
between gains is high. Fine- to medium-grained sands do not allow
rapid draining of pore water but have void spaces large enough to
make capillary cohesion irrelevant. These sand sizes are common
in recently deposited marine and river sediments, and so liquefaction
commonly occurs in these materials during earthquakes (Bryant 1991,
203-206).
Liquefaction is represented by lateral spreads (movement of blocks
of soil over a liquefied layer beneath the surface), flow failures
(fluidised soils moving down slope as a slurry) and loss of bearing
strength. During the 1906 San Francisco earthquake, liquefaction
spreads in estuarine muds and landfill near the harbour broke water
mains, hampering fire fighting. Liquefaction was also a problem
during the 27 March 1964 Alaskan earthquake where nearly every bridge
in the affected area was destroyed by lateral spreads. On 16 June,
1964 a major earthquake struck 60 km north of Niigata city, Japan.
The earthquake produced maximum ground accelerations of 1.58 cm
s-2 (i.e. about MM II, not excessive). The earthquake,
however, liquefied river sediments on Shinano River, across which
the city had expanded, and large apartment blocks sank into the
liquefied sediments.
Although liquefaction generally occurs in water-saturated soils,
it can also involve air. During the 16 December 1920 Kansu earthquake,
200,000 people were buried by landslides triggered by liquefaction
of fine-grained loess. The shear strength of the loess was exceeded
by the earthquake effects but low permeability of the fine-grained
soil prevented air from escaping from the loess, which then liquefied.
Tsunami
A tsunami is a series of large ocean waves generated
by impulses from geophysical events, such as earthquakes, landslides
and volcanic eruptions, on the ocean bottom or along the coastline.
The word Tsunami was coined by the Japanese and translates literally
to "great wave in harbour".
The most common event that generates tsunamis is submarine faulting
which causes part of the ocean floor to be vertically displaced.
Only a small number of submarine earthquakes result in tsunamis.
Most have a small spatial effect or are too low in magnitude to
cause vertical displacement of the ocean floor. Generally, only
those earthquakes with a Richter magnitude > 6.6, and shallow
focal depths (< 50 km) are likely to be accompanied by tsunamis.
The abrupt submarine faulting and vertical displacement of the sea
floor causes rapid upward movement of the overlying water column,
forcing it into a wave-like turbulence, which is expressed as a
series of seismic sea waves. In the ocean these are barely detectable
and difficult to tell from normal swells. They have low wave heights
(~ 1 m) and long wavelengths (100 km) and travel at speeds up to
1000 km/hr. A tsunami may comprise numerous waves, which may arrive
at the shoreline minutes or hours apart.
The April 1946 Alaskan earthquake triggered a tsunami which damaged
coastal areas in the Aleutian Islands, and also crossed the Pacific
Ocean to strike Hilo Bay in Hawaii. A 30-m high wave, travelling
at approximately 780 km/hr, killed five people. At Hilo Bay tsunami
waves crested more than 19 m above normal water level, and waves
swept more than 600 m inland. Some 500 homes and businesses were
destroyed and thousands damaged; 96 people were killed. Hawaii was
also affected by tsunamis following the Richter magnitude 8.6, 22
May 1960 Chilean earthquake, which generated a Pacific-wide tsunami.
Tsunamis and their effects are fully explored in another module.
|